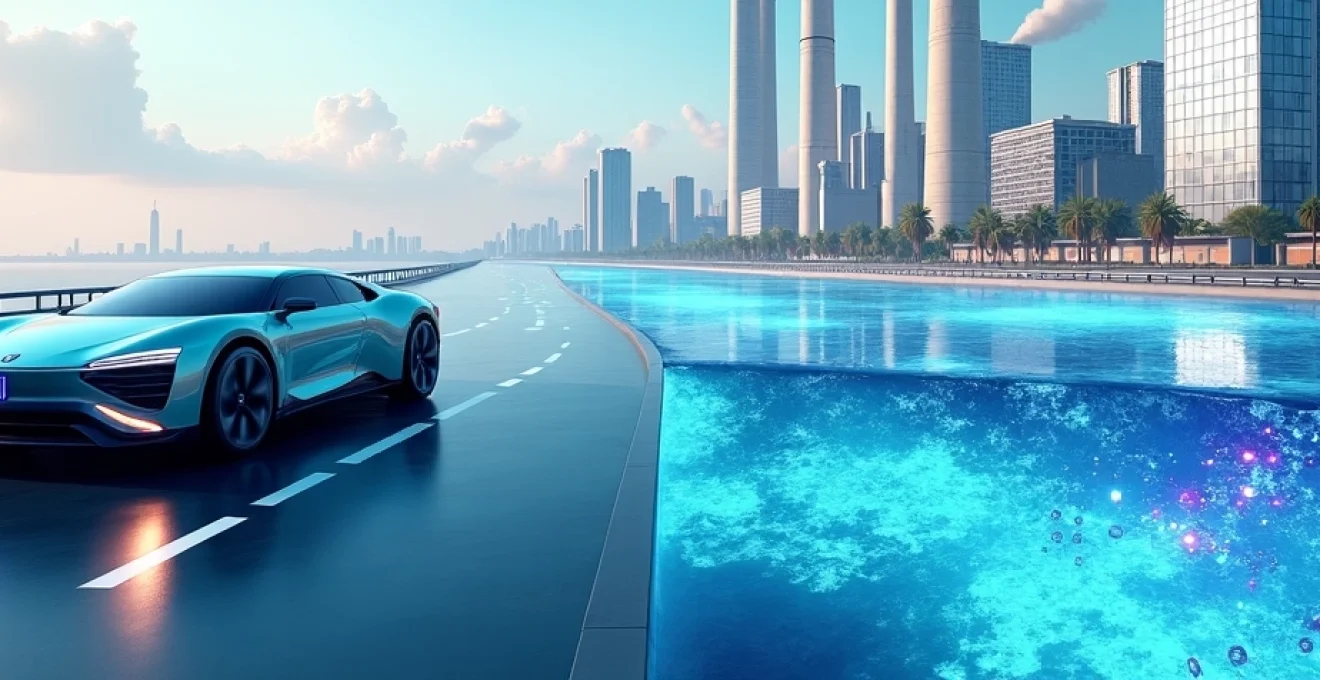
Hydrogen is rapidly emerging as a versatile and promising solution for the global transition to clean energy. As countries worldwide strive to reduce carbon emissions and combat climate change, hydrogen's potential applications span from large-scale energy storage to powering zero-emission vehicles. This clean energy carrier offers unique advantages in its ability to store renewable energy, provide grid stability, and enable clean transportation across various sectors. With advancements in production methods, storage technologies, and fuel cell systems, hydrogen is poised to play a crucial role in shaping a sustainable energy future.
Hydrogen production methods for energy applications
The production of hydrogen is a critical component in realizing its potential as a clean energy carrier. Various methods exist for hydrogen production, each with its own set of advantages and challenges. As the demand for clean hydrogen grows, innovative technologies are being developed to increase efficiency and reduce costs. Let's explore some of the most promising hydrogen production methods for energy applications.
Electrolysis: Proton Exchange Membrane (PEM) technology
Proton Exchange Membrane (PEM) electrolysis is at the forefront of green hydrogen production. This technology uses electricity to split water molecules into hydrogen and oxygen, with no carbon emissions if powered by renewable energy sources. PEM electrolyzers offer several advantages, including rapid response times, high efficiency, and the ability to produce ultra-pure hydrogen. You'll find that PEM technology is particularly well-suited for integration with intermittent renewable energy sources like wind and solar power.
Recent advancements in PEM electrolysis have led to significant cost reductions and improved durability. For instance, researchers have developed new catalyst materials that reduce the need for expensive precious metals like platinum. This progress is crucial for scaling up green hydrogen production and making it economically competitive with fossil fuel-derived hydrogen.
Steam Methane Reforming (SMR) and carbon capture
Steam Methane Reforming (SMR) remains the most widely used method for large-scale hydrogen production. This process involves reacting natural gas with high-temperature steam to produce hydrogen and carbon dioxide. While SMR is currently the most cost-effective method, it relies on fossil fuels and generates significant CO2 emissions. To address this environmental concern, carbon capture and storage (CCS) technologies are being integrated with SMR facilities.
The combination of SMR with CCS, often referred to as "blue hydrogen," offers a transitional solution towards cleaner hydrogen production. You can expect to see more SMR facilities incorporating advanced carbon capture technologies in the coming years, as industries work to reduce the carbon footprint of hydrogen production while scaling up capacity to meet growing demand.
Thermochemical water splitting using solar energy
Thermochemical water splitting represents an innovative approach to hydrogen production that harnesses the power of concentrated solar energy. This method uses high temperatures generated by concentrated solar collectors to drive a series of chemical reactions that ultimately split water into hydrogen and oxygen. The process typically involves multiple steps and utilizes specialized materials that can withstand extreme temperatures.
One of the main advantages of thermochemical water splitting is its potential for high efficiency and the ability to produce hydrogen without electricity or fossil fuels. However, challenges remain in developing durable materials that can withstand the harsh conditions of the process and in scaling up the technology for commercial applications. As research progresses, you may see this method becoming more viable for large-scale hydrogen production in regions with abundant solar resources.
Biological hydrogen production: algal and bacterial processes
Biological hydrogen production methods offer a fascinating glimpse into the potential for sustainable, nature-inspired hydrogen generation. These processes leverage the metabolic capabilities of certain microorganisms to produce hydrogen as a byproduct of their growth. Two primary approaches in this field are algal hydrogen production and dark fermentation using bacteria.
Algal hydrogen production utilizes certain species of green algae that can produce hydrogen through photobiological processes. Under specific conditions, these algae can switch from producing oxygen to producing hydrogen. While this method is still in the early stages of development, it holds promise for sustainable hydrogen production using only sunlight and water.
Dark fermentation, on the other hand, employs anaerobic bacteria to break down organic matter and produce hydrogen as a metabolic byproduct. This process has the added benefit of potentially utilizing organic waste streams, contributing to waste management solutions while generating clean energy. As research in biological hydrogen production advances, you might see these methods playing a complementary role in the hydrogen economy, particularly in regions with suitable climates and abundant biomass resources.
Hydrogen storage technologies for grid-scale applications
As hydrogen production scales up to meet growing demand, the need for efficient and large-scale storage solutions becomes increasingly critical. Grid-scale hydrogen storage is essential for balancing supply and demand, integrating renewable energy sources, and ensuring a stable energy supply. Several technologies are being developed and implemented to address the unique challenges of storing hydrogen at scale.
Compressed hydrogen storage in salt caverns
Salt caverns offer a promising solution for large-scale hydrogen storage. These naturally occurring or artificially created caverns in underground salt formations can store vast quantities of compressed hydrogen gas. The impermeability of salt to hydrogen makes these structures ideal for long-term storage with minimal leakage.
You'll find that salt cavern storage is particularly attractive for its scalability and low cost per unit of energy stored. Several projects in Europe are already utilizing this technology, with plans for expansion as hydrogen production increases. The ability to store hydrogen seasonally in salt caverns could play a crucial role in managing the intermittency of renewable energy sources and ensuring a stable supply of clean energy throughout the year.
Liquid hydrogen cryogenic storage systems
Liquid hydrogen storage offers significant advantages in terms of energy density, making it an attractive option for applications where space is at a premium. To liquefy hydrogen, it must be cooled to extremely low temperatures of around -253°C (-423°F). While this process requires substantial energy input, the resulting liquid hydrogen can be stored more compactly than compressed gas.
Cryogenic storage systems for liquid hydrogen are being developed for both stationary and mobile applications. In the context of grid-scale storage, large insulated tanks can hold vast quantities of liquid hydrogen for extended periods. However, challenges remain in minimizing boil-off losses and improving the energy efficiency of the liquefaction process. As these technologies advance, you may see liquid hydrogen storage playing an increasingly important role in the hydrogen supply chain, particularly for long-distance transport and high-demand applications.
Metal hydride storage for compact applications
Metal hydride storage represents an innovative approach to hydrogen storage that offers high volumetric density and enhanced safety. This technology relies on the ability of certain metals and alloys to absorb hydrogen atoms into their crystal structure, forming stable metal hydrides. When heat is applied, the hydrogen is released, allowing for controlled and reversible storage.
While currently more suitable for smaller-scale applications due to weight considerations, ongoing research is exploring the potential of metal hydride storage for larger systems. The compact nature of metal hydride storage makes it particularly attractive for mobile applications and situations where space is limited. As new materials with improved hydrogen storage capacities are developed, you might see metal hydride systems finding niche applications in grid-scale storage, particularly for rapid response and short-duration needs.
Underground Hydrogen Storage (UHS) in depleted gas fields
Underground Hydrogen Storage (UHS) in depleted gas fields presents a promising option for large-scale, long-term hydrogen storage. This method repurposes existing infrastructure from the natural gas industry, potentially offering a cost-effective solution for storing vast quantities of hydrogen. Depleted gas fields have proven geological seals and well-understood characteristics, making them attractive candidates for hydrogen storage.
The concept of UHS in depleted gas fields is similar to that of natural gas storage, but it comes with unique challenges due to hydrogen's properties. Research is ongoing to address issues such as potential hydrogen leakage, interactions with reservoir rocks and microorganisms, and the development of appropriate monitoring systems. As the hydrogen economy grows, you can expect to see increased interest in UHS as a vital component of the hydrogen infrastructure, particularly in regions with suitable geological formations and existing gas industry expertise.
Fuel cell systems for clean mobility solutions
Fuel cell technology is revolutionizing the transport sector by offering a clean, efficient alternative to traditional combustion engines. As concerns over air quality and carbon emissions grow, fuel cell systems are gaining traction across various modes of transportation. These systems convert hydrogen into electricity, with water vapor as the only byproduct, making them an attractive option for zero-emission mobility.
Proton Exchange Membrane Fuel Cells (PEMFC) in automotive applications
Proton Exchange Membrane Fuel Cells (PEMFCs) have emerged as the leading technology for fuel cell vehicles. These systems offer high power density, quick start-up times, and excellent dynamic response, making them well-suited for automotive applications. Major automakers have already introduced PEMFC-powered vehicles to the market, with more models expected in the coming years.
One of the key advantages of PEMFCs is their ability to provide long driving ranges comparable to conventional vehicles, while offering rapid refueling times. This addresses two major concerns associated with battery electric vehicles: range anxiety and long charging times. As hydrogen refueling infrastructure expands, you'll likely see increased adoption of PEMFC vehicles, particularly in segments where long range and quick refueling are critical, such as taxis, delivery fleets, and long-haul trucking.
Solid Oxide Fuel Cells (SOFC) for Heavy-Duty transport
Solid Oxide Fuel Cells (SOFCs) are gaining attention for their potential in heavy-duty transport applications. These high-temperature fuel cells offer higher electrical efficiency compared to PEMFCs and can utilize a variety of fuels, including hydrogen, natural gas, and biogas. While traditionally used in stationary power generation, advancements in materials and design are making SOFCs increasingly viable for mobile applications.
The high efficiency and fuel flexibility of SOFCs make them particularly attractive for long-haul trucking and maritime applications. These sectors require high power output and long operating hours, which align well with the characteristics of SOFC systems. As SOFC technology continues to mature, you may see it playing a significant role in decarbonizing heavy-duty transport, complementing battery electric and PEMFC solutions in the quest for clean mobility.
Alkaline Fuel Cells (AFC) in aerospace and marine sectors
Alkaline Fuel Cells (AFCs) have a long history in aerospace applications, having been used in NASA's space programs since the 1960s. These fuel cells offer high efficiency and can operate at lower temperatures compared to SOFCs. While less common in terrestrial applications due to sensitivity to CO2, AFCs are finding renewed interest in specialized sectors such as aerospace and marine applications.
In the aerospace industry, AFCs continue to be used in certain spacecraft and satellite systems due to their reliability and the ability to produce potable water as a byproduct. In the marine sector, AFCs are being explored for use in submarines and other vessels where air-independent propulsion is desirable. As research progresses, you might see AFCs carving out niche applications in these specialized mobility sectors, contributing to the diversification of fuel cell technologies in clean transport solutions.
Microbial fuel cells (MFC) for wastewater treatment and energy recovery
Microbial Fuel Cells (MFCs) represent an innovative intersection of biotechnology and clean energy production. These systems utilize microorganisms to break down organic matter in wastewater, generating electricity in the process. While not typically associated with mobility applications, MFCs offer unique potential for sustainable energy recovery in wastewater treatment facilities.
The development of MFC technology could lead to interesting applications in the broader context of clean mobility. For instance, wastewater treatment plants equipped with MFCs could potentially generate hydrogen or electricity to power municipal vehicle fleets. This synergistic approach to waste management and clean energy production exemplifies the kind of integrated solutions needed for a sustainable future. As research in this field advances, you may see MFCs contributing to localized, circular energy systems that support clean mobility initiatives in urban environments.
Hydrogen infrastructure and distribution networks
The development of robust hydrogen infrastructure and distribution networks is crucial for the widespread adoption of hydrogen as a clean energy carrier. As hydrogen production scales up and demand grows across various sectors, efficient and safe methods for transporting and distributing hydrogen are becoming increasingly important. This infrastructure challenge requires significant investment and coordination among stakeholders to create a comprehensive hydrogen economy.
One of the key components of hydrogen infrastructure is the establishment of a network of hydrogen refueling stations. These stations are essential for supporting the growth of fuel cell vehicles and ensuring that hydrogen is readily available for consumers. You'll find that countries like Japan, Germany, and California are leading the way in deploying hydrogen refueling stations, with plans for significant expansion in the coming years.
In addition to refueling stations, the development of hydrogen pipelines is crucial for large-scale distribution. While some existing natural gas pipelines can be repurposed for hydrogen transport, dedicated hydrogen pipelines are being planned and constructed in various regions. These pipelines will enable the efficient transport of hydrogen from production sites to end-users, supporting both industrial applications and clean mobility solutions.
Another important aspect of hydrogen infrastructure is the creation of storage hubs and distribution centers. These facilities will play a vital role in balancing supply and demand, ensuring a stable hydrogen supply chain. As the hydrogen economy grows, you can expect to see the emergence of regional hydrogen hubs that integrate production, storage, and distribution capabilities to serve diverse end-users efficiently.
Safety protocols and regulations in hydrogen handling
As hydrogen adoption increases across various sectors, ensuring safe handling and use of this clean energy carrier is paramount. Comprehensive safety protocols and regulations are being developed and implemented to address the unique properties of hydrogen and mitigate potential risks associated with its production, storage, and use.
One of the primary safety considerations in hydrogen handling is its high flammability and wide range of combustible concentrations in air. To address this, stringent safety standards have been established for hydrogen storage systems, including requirements for ventilation, leak detection, and fire suppression. You'll find that these standards are continually evolving as new technologies and applications emerge, with international collaboration playing a crucial role in developing best practices.
In the context of hydrogen fuel cell vehicles, safety regulations cover aspects such as crash testing, fuel system integrity, and emergency shutdown procedures. Automakers are incorporating advanced safety features into their fuel cell vehicles, including sensors that can detect hydrogen leaks and automatically shut off the fuel supply. As the number of hydrogen vehicles on the roads increases, public awareness and education about hydrogen safety will become increasingly important.
For industrial applications and large-scale hydrogen facilities, comprehensive risk assessment methodologies are being developed to identify and mitigate potential hazards. These assessments consider factors such as facility design, operational procedures, and emergency response plans. Regular inspections and maintenance protocols are also crucial for ensuring the ongoing safety of hydrogen infrastructure.
Economic viability and market projections for hydrogen technologies
The economic viability of hydrogen technologies is a critical factor in determining their widespread adoption and impact on the global energy landscape. As production scales up and technologies mature, the cost of hydrogen is expected to decrease significantly, making it more competitive with traditional energy sources.
Current projections indicate that the global hydrogen market could reach a value of $200 billion by 2030, driven by increasing demand in sectors such as transportation, industry, and power generation. You'll find that government support and private sector investment are playing crucial roles in accelerating the development and deployment of hydrogen technologies.
One of the key economic drivers for hydrogen adoption is the declining cost of renewable electricity, which is essential for producing green hydrogen through electrolysis. As renewable energy costs continue to fall, the production of clean hydrogen is becoming increasingly cost-competitive. Some analysts predict that green hydrogen could reach price parity with fossil fuel-derived hydrogen in certain regions by 2030.
In the mobility sector, the total cost of ownership for fuel cell vehicles is expected to decrease as production volumes increase and fueling infrastructure expands. While currently more expensive than conventional vehicles, fuel cell cars and buses are projected to become cost-competitive in the coming years, particularly in markets with supportive policies and incentives.
As the hydrogen economy grows, new business models and value chains are emerging. From hydrogen production and distribution to end-use applications, opportunities are arising for companies to participate in this evolving market. The development of integrated projects that combine renewable energy production, hydrogen generation, and various end-use applications is likely to drive economies of scale and improve overall economic viability.
While challenges remain in scaling up hydrogen technologies and reducing costs across the value chain, the long-term economic prospects for hydrogen are promising. As governments worldwide commit to decarbonization targets and industries seek clean energy solutions, hydrogen is poised to play a significant role in the global energy transition, offering both environmental benefits and economic opportunities.